The Molecular Biology of Memory Storage: A Dialogue Between Genes and Synapses
Kandel, Science 2001
- One of the most remarkable aspects of an animal’s behavior is the ability to modify that behavior by learning, an ability that reaches its highest form in human beings
- For me, learning and memory have proven to be endlessly fascinating mental processes because they address one of the fundamental features of human activity: our ability to acquire new ideas from experience and to retain these ideas over time in memory
- Moreover, unlike other mental processes such as thought, language, and consciousness, learning seemed from the outset to be readily accessible to cellular and molecular analysis
- I, therefore, have been curious to know: What changes in the brain when we learn? And, once something is learned, how is that information retained in the brain? I have tried to address these questions through a reductionist approach that would allow me to investigate elementary forms of learning and memory at a cellular molecular level—as specific molecular activities within identified nerve cells.
Figure 1 - A Simple Learned Behavior
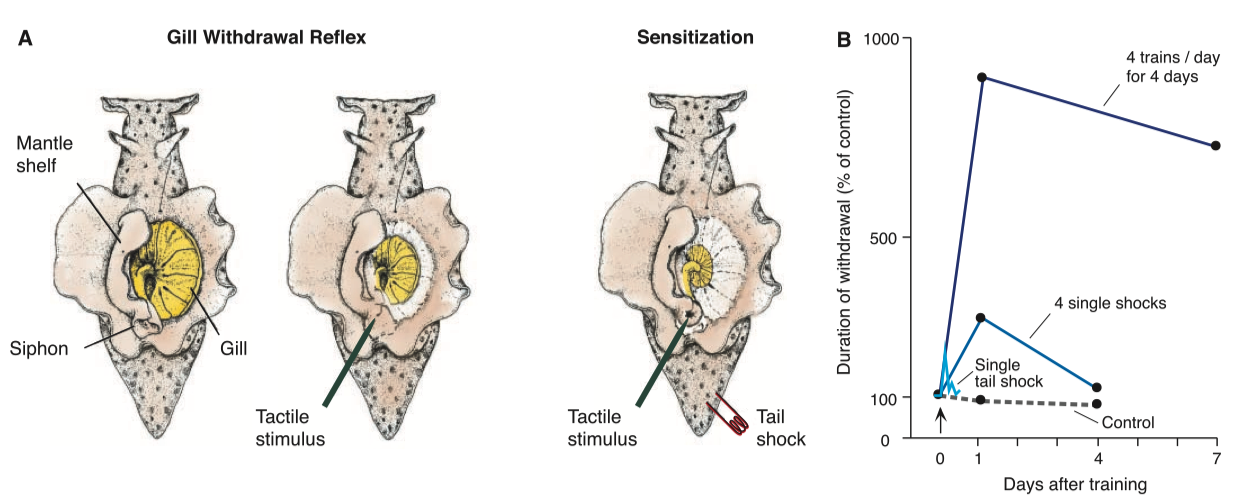
- (A) A dorsal view of Aplysia showing the gill, the animal’s respiratory organ
- A light touch to the siphon with a fine probe causes the siphon to contract and the gill to withdraw
- Here, the mantle shelf is retracted for a better view of the gill
- Sensitization of the gill-withdrawal reflex, by applying a noxious stimulus to another part of the body, such as the tail, enhances the withdrawal reflex of both the siphon and the gill
- (B) Spaced repetition converts short-term memory into long-term memory in Aplysia
- Before sensitization training, a weak touch to the siphon causes only a weak, brief siphon and gill withdrawal reflex
- Following a single noxious, sensitizing, shock to the tail, that same weak touch produces a much larger siphon and gill reflex withdrawal response, an enhancement that lasts about 1 hour
- More tail shocks increase the size and duration of the response
- [Modified from (79)]
Figure 2 - The Neural Circuit of the Aplysia Gill-Withdrawal Reflex
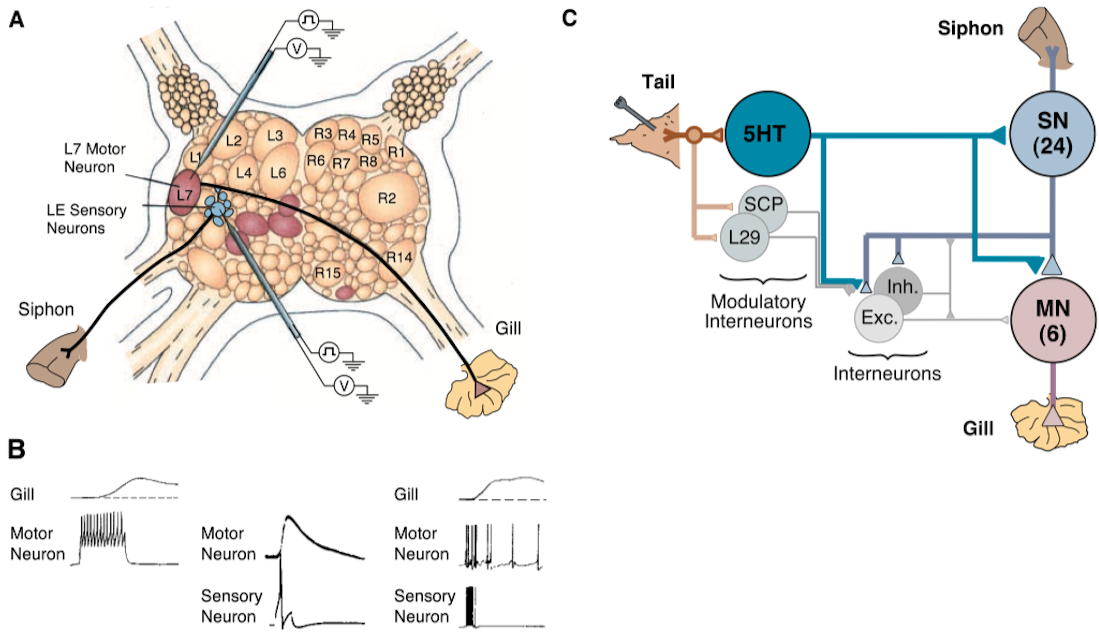
- (A) In this dorsal view of the abdominal ganglion, the six identified motor cells to the gill are brown and the seven sensory neurons are blue
- A sensory neuron that synapses on gill motor neuron L7 is stimulated electrically with an intracellular electrode and a microelectrode in the motor neuron records the synaptic potential produced by the action potential in the sensory neuron [see middle trace in (B)]
- The sensory neuron carries the input from the siphon skin; the motor neuron makes direct connections onto the gill
- (B) Individual cells make significant contributions to the reflex
- Stimulating a single motor neuron (traces on the left) produces a detectable change in the gill and stimulating a single sensory neuron produces a large synaptic potential in the motor neuron (traces in the middle)
- Repeated stimulation of a single sensory neuron increases the frequency of firing in the motor neuron, leading to a visible reflex contraction of the gill (traces on the right)
- A single tactile stimulus to the skin normally activates 6 to 8 of the 24 sensory neurons, causing each to fire 1 to 2 action potentials
- The repetitive firing of 10 action potentials in a single sensory neuron, designed to simulate the firing of the total population (trace on the right) simulates the reflex behavior reasonably well
- (C) Diagram of the circuit of the gill-withdrawal reflex
- The siphon is innervated by 24 sensory neurons that connect directly with the six motor neurons
- The sensory neurons also connect to populations of excitatory and inhibitory interneurons that in turn connect with the motor neurons
- Stimulating the tail activates three classes of modulatory interneurons (serotonergic neurons, neurons that release the small cardioactive peptide, and the L29 cells) that act on the terminals of the sensory neurons as well as on those of the excitatory interneurons
- The serotonergic modulatory action is the most important; blocking the action of these cells blocks the effects of sensitizing stimuli [From (25)]
Intro
- I first became interested in the study of memory in 1950 as a result of my readings in psychoanalysis while still an undergraduate at Harvard College
- Later, during medical training, I began to find the psychoanalytic approach limiting because it tended to treat the brain, the organ that generates behavior, as a black box
- In the mid-1950s, while still in medical school, I began to appreciate that during my lifetime the black box of the brain would be opened and that the problems of memory storage, once the exclusive domain of psychologists and psychoanalysts, could be investigated with the methods of modern biology
- As a result, my interest in memory shifted from a psychoanalytic to a biological approach
- As a postdoctoral fellow at the National Institutes of Health (NIH) in Bethesda from 1957 to 1960, I focused on learning more about the biology of the brain and became interested in knowing how learning produces changes in the neural networks of the brain
- My purpose in translating questions about the psychology of learning into the empirical language of biology was not to replace the logic of psychology or psychoanalysis with the logic of cellular molecular biology, but to try to join these two disciplines and to contribute to a new synthesis that would combine the mentalistic psychology of memory storage with the biology of neuronal signaling
- I hoped further that the biological analysis of memory might carry with it an extra bonus, that the study of memory storage might reveal new aspects of neuronal signaling
- Indeed, this has proven true.
A Radical Reductionist Strategy to Learning and Memory
- At first thought, someone interested in learning and memory might be tempted to tackle the problem in its most complex and interesting form
- This was the approach that Alden Spencer and I took when we joined forces at NIH in 1958 to study the cellular properties of the hippocampus, the part of the mammalian brain thought to be most directly involved in aspects of complex memory (1)
- We initially asked, rather naı¨vely: Are the electrophysiological properties of the pyramidal cells of the hippocampus, which were thought to be the key hippocampal cells involved in memory storage, fundamentally different from other neurons in the brain? With study, it became clear to us that all nerve cells, including the pyramidal cells of the hippocampus, have similar signaling properties
- Therefore, the intrinsic signaling properties of neurons would themselves not give us key insights into memory storage (2)
- The unique functions of the hippocampus had to arise not so much from the intrinsic properties of pyramidal neurons but from the pattern of functional interconnections of these cells, and how those interconnections are affected by learning
- To tackle that problem we needed to know how sensory information about a learning task reaches the hippocampus and how information processed by the hippocampus influences behavioral output
- This was a formidable challenge, since the hippocampus has a large number of neurons and an immense number of interconnections
- It seemed unlikely that we would be able to work out in any reasonable period of time how the neural networks, in which the hippocampus was embedded, participate in behavior and how those networks are affected by learning
- To bring the power of modern biology to bear on the study of learning, it seemed necessary to take a very different approach—a radically reductionist approach
- We needed to study not the most complex but the simplest instances of memory storage, and to study them in animals that were most tractable experimentally
- Such a reductionist approach was hardly new in 20th-century biology
- One need only think of the use of Drosophila in genetics, of bacteria and bacteriophages in molecular biology, and of the squid giant axon in the study of the conduction of nerve impulses
- Nevertheless, when it came to the study of behavior, many investigators were reluctant to use a reductionist strategy
- In the 1950s and 1960s many biologists and most psychologists believed that learning was the one area of biology in which the use of simple animal models, particularly invertebrate ones, was least likely to succeed
- They argued that only higher animals exhibit interesting forms of learning and that these forms require neuronal organizations and neuronal mechanisms qualitatively different from those found in simple animals
- It was my belief, however, that concerns about the use of a simple experimental system to study learning were misplaced
- If elementary forms of learning are common to all animals with an evolved nervous system, there must be conserved features in the mechanisms of learning at the cell and molecular level that can be studied effectively even in simple invertebrate animals.
A Simple Learned Behavior in an Invertebrate
- After an extensive search for a suitable experimental animal, I settled on the giant marine snail Aplysia (Fig
- 1A) because it offers three important advantages: Its nervous system is made up of a small number of nerve cells; many of these are gigantic; and (as became evident to me later) many are uniquely identifiable (3, 4)
- Whereas the mammalian brain has a trillion central nerve cells, Aplysia has only 20,000, and the simplest behaviors that can be modified by learning may directly involve less than 100 central nerve cells
- In addition to being few in numbers, these cells are the largest nerve cells in the animal kingdom, reaching up to 1000 m m in diameter, large enough to be seen with the naked eye
- One can record from these large cells for many hours without any difficulty, and the same cell can be returned to and recorded from over a period of days
- The cells can easily be dissected out for biochemical studies, so that from a single cell one can obtain sufficient mRNA to make a cDNA library
- Finally, these identified cells can readily be injected with labeled compounds, antibodies, or genetic constructs, procedures which opened up the molecular study of signal transduction within individual nerve cells
- Irving Kupfermann and I soon delineated a very simple defensive reflex: The withdrawal of the gill upon stimulation of the siphon, an action that is like the quick withdrawal of a hand from a hot object
- When a weak tactile stimulus is applied to the siphon, both the siphon and gill are withdrawn into the mantle cavity for protection under the mantle shelf (Fig. 1A) (5)
- Kupfermann, Harold Pinsker, and later Tom Carew, Robert Hawkins, and I found that this simple reflex could be modified by three different forms of learning: habituation, sensitization, and classical conditioning (5–7)
- As we examined these three forms of learning, we were struck by the resemblance each had to corresponding forms of learning in higher vertebrates and humans
- As with vertebrate learning, memory storage for each type of learning in Aplysia has two phases: a transient memory that lasts minutes and an enduring memory that lasts days
- Conversion of short-term to long-term memory storage requires spaced repetition—practice makes perfect, even in snails (Fig. 1B) (6–8)
- We focused initially on one type of learning
- Sensitization is a form of learned fear in which a person or an experimental animal learns to respond strongly to an otherwise neutral stimulus (5, 6, 8)
- For example, if a person is suddenly exposed to an aversive stimulus, such as a gunshot going off nearby, that person will be sensitized by the unexpected noise
- As a result, that person will be frightened and will now startle to an otherwise innocuous stimulus like a tap on the shoulder
- Similarly, on receiving an aversive shock to a part of the body such as the tail, an Aplysia recognizes the stimulus as aversive and learns to enhance its defensive reflex responses to a variety of subsequent stimuli applied to the siphon, even innocuous stimuli (Fig. 1A) (9)
- The animal remembers the shock, and the duration of this memory is a function of the number of repetitions of the noxious experience (Fig. 1B)
- A single shock gives rise to a memory lasting only minutes; this short-term memory does not require the synthesis of new protein
- In contrast, four or five spaced shocks to the tail give rise to a memory lasting several days; this long-term memory does require the synthesis of new protein
- Further training, four brief trains a day for four days, gives rise to an even more enduring memory lasting weeks, which also requires new protein synthesis
- Thus, just as in complex learning in mammals (10, 11), the long-term memory for sensitization differs from the short-term memory in requiring the synthesis of new proteins
- This was our first clear evidence for the conservation of biochemical mechanisms between Aplysia and vertebrates
- Kupfermann, Castellucci, Carew, Hawkins, John Byrne, and I worked out significant components of the neural circuit gill-withdrawal reflex (Fig. 2)
- The circuit is located in the abdominal ganglion and has 24 central mechanoreceptor sensory neurons that innervate the siphon skin and make direct monosynaptic connections with 6 gill motor cells (Fig. 2C) (12–14)
- The sensory neurons also made indirect connections with the motor cells through small groups of excitatory and inhibitory interneurons (15, 16)
- In addition to being identifiable, individual cells also have surprisingly large effects on behavior (Fig. 2B) (4, 14, 17)
- As we examined the neural circuit of this reflex, we were struck by its invariance
- In every animal we examined, each cell connected only to certain target cells and not to others (Fig. 2C)
- This also was true in the neural circuitry of other behaviors in Aplysia including inking, control of the circulation, and locomotion (4, 18)
- This raised a key question in the cell biological study of learning: How can learning occur in a neural circuit that is so precisely wired? In 1894, Santiago Ramo´n y Cajal proposed a theory of memory storage according to which memory is stored in the growth of new connections (19)
- This prescient idea was neglected in good part for half a century as students of learning fought over newer competing ideas
- First, Karl Lashley, Wolfgang Ko¨hler, and a number of Gestalt psychologists proposed that learning leads to changes in electric fields or chemical gradients, which they postulated surround neuronal populations and are produced by the aggregate activity of cells recruited by the learning process
- Second, Alexander Forbes and Lorente de No´proposed that memory is stored dynamically by a self-reexciting chain of neurons
- Donald Hebb later championed this idea as a mechanism for short-term memory
- Finally, Holger Hyden proposed that learning led to changes in the base composition of DNA or RNA
- Even though there was much discussion about the merits of each of these ideas, there was no direct evidence to support any of them (2)
- Kupfermann, Castellucci, Carew, Hawkins, and I addressed these alternative ideas directly by confronting the question of how learning can occur in a circuit with fixed neuronal elements
- To address this question, we examined the neural circuit of the gill-withdrawal reflex while the animal underwent sensitization, classical conditioning, or habituation
- Our studies provided clear evidence for the idea proposed by Ramo´n y Cajal, that learning results from changes in the strength of the synaptic connections between precisely interconnected cells (12, 20)
- Thus, while the organism’s developmental program assures that the connections between cells are invariant, it does not specify their precise strength
- Rather, experience alters the strength and effectiveness of these preexisting chemical connections
- Seen in the perspective of these three forms of learning, synaptic plasticity emerged as a fundamental mechanism for information storage by the nervous system, a mechanism that is built into the very molecular architecture of chemical synapses (21).